Correlating Charmed and Light Mesons in Electron-Proton Scattering
ABSTRACT
Within an atom exists protons, electrons, and neutrons. Within a proton there are two particles: quarks and gluons. It is crucial to understand the beginning of the Universe to predict its evolution. This is the role of high energy physics facilities such as CERN (large hadron collider) and the Brookhaven Laboratory (RHIC and EIC) enabling us to break open protons. The goal of particle physics is to study the particles that come out of these collisions. Two of these are the D0 meson and the charged Pion. The D0 is made up of a charm quark and an antiup quark, and the Pion is made up of an up quark and an antidown quark. Using PYTHIA 8, a program that enables us to simulate collisions at the large hadron colliders and changing the virtual photon energy (Q2) it was found that the overall energy increased along with the D0 and the Pion’s individual transverse momentums (pτ). We found that using an increased virtual photon energy increased the overall energy and pτ of the reaction products, as expected, and we could gauge the ability of the RHIC to carry out this research.
INTRODUCTION.
Particle physics studies the smallest particles and strives to understand how these particles interact with each other. Particle physics is relevant to how everything in the Universe works and exists. The overarching questions all particle physicists strive to answer are “What is inside of a proton?” and “How do these particles interact to create the Universe we live in everyday?”
Inside of a Proton.
Quarks and gluons are the fundamental particles that make up a proton [6]. Gluons hold quarks together inside of the proton using the strong force (one of the four fundamental forces in nature including gravity, electromagnetism and the weak force [4]). Following the Big Bang, a quark-gluon plasma formed and expanded, creating the Universe and everything in it. If we can understand the quark-gluon plasma, then it will be easier to understand how things interact currently in the Universe based on what we learn from its formation.
Within a proton there are many different flavors of quarks and gluons that are interacting to create the positively charged proton. A meson is a particle made up of both a quark and an antiquark. Specifically, the D0 meson is made up of two types of quarks including the charm quark (m = 1.27 GeV) and an antiup quark (m = 2.16 MeV). The variable m meaning the rest mass of the particle, the mass of the particle when at rest. The units used to measure the resting mass of particles is GeV which are the giga-electron volts of the specific particle. The pion is made up of an up quark (m = 2.16 MeV) and an antidown quark (m = 4.67 MeV)
Antimatter is one of the mysteries of the Universe. To best understand the use and existence of antimatter there is a metaphor: When you dig a hole on a beach there will be a pile of sand and there will be a hole. The pile of dirt would be all the matter we see around us in the world and the known Universe, but the hole would be antimatter. When these two (matter and antimatter) are brought together there is an emission of light. Because the D0 is made up of a charm quark and an antiup quark it is unstable. The two opposing particles will decay into a more stable element quickly after the collision. This makes it incredibly difficult to study these particles. The job of a physicist is to take the data collected by these colliders and retrace the paths or tracks of the particles back into the proton and understand how they behaved before and during the collision. Essentially this experiment simulates these collisions to have a better understanding of the expected outcome before the collisions commence.
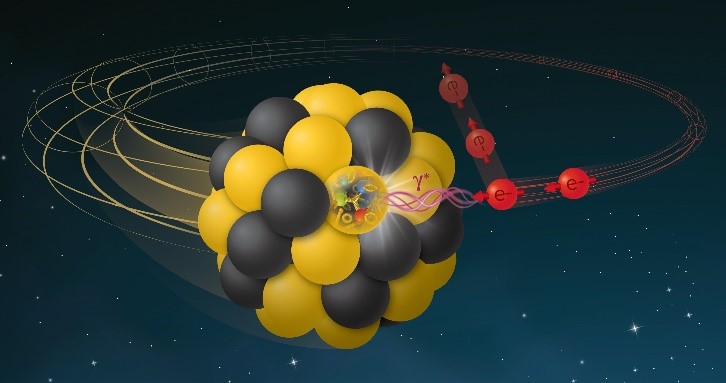
Transverse Momentum (pτ).
Electron-proton collisions take place between the virtual photon emitted by the electron and proton within the atomic nucleus (nucleus of the atom). This virtual photon energy is represented by Q2 charge in GeV[2]. The energy necessary to emit the photon sends the electron scattering in the opposite direction following Newton’s law of reaction and momentum conservation explaining the term electron scattering.
To record and understand how these particles move and decay within the natural environment, the transverse momentum of the particles are recorded. Transverse momentum (pτ) is the momentum of the particles as they are tracked through the detector within the XY plane where the Z axis is the direction of the beam.
Existing Colliders and Future Directions.
There are two existing colliders in the world currently: LHC (Large Hadron Collider) and the Relativistic Heavy Ion Collider (RHIC). The collider located on the Franco-Swiss border near Geneva Switzerland is the LHC. The physical collider is 100 km under the Earth to protect the public from the large amount of radiation due to the nuclear collisions happening within the LHC collider. CERN stands for Conseil Européen pour la Recherché Nucléaire, or the European Council for Nuclear Research. CERN collects data from these collisions and sends it to be analyzed. Vanderbilt University is one of the locations where data is analyzed by the Nuclear Physics group.
The way that these collisions take place is by the manipulation of electromagnetic fields. Because protons have a charge, they can be manipulated by an electromagnetic field to then move them at such a high acceleration that when run into each other they break open exposing what is inside. The Large Hadron Collider consists of a 27 km ring of superconducting magnets with several accelerating structures meant to rapidly increase the speed of the particles. Built in 2008, this collider has been running and collecting data on proton-proton collisions [5].
These collisions are different from those set to happen in the EIC (Electron-Ion Collider). Like the LHC, there is also a Relativistic Heavy Ion Collider (RHIC) at Brookhaven National Laboratory (BNL) Upton, New York. While smaller than the hadron collider, BNL created this collider to accelerate more varieties of elements. Primarily the RHIC uses Gold Ions which are Heavy Ions. Like RHIC, it has made plans to create an Electron-Ion Collider (EIC) within the next 10 years [6]. By 2033 the EIC will collide protons and electrons in stark comparison to the other two colliders tasked with colliding protons with protons which is what my data is preliminary research for.
When a proton collides with another proton there is a large spray of quarks and gluons that are ejected from the collision. However, this makes it difficult to understand where these particles exist within each proton prior to colliding. Electron-proton collisions will be able to identify where the quarks and gluons are located within the proton [3]. Because electrons won’t break open like a proton this collision will be easier to identify where each subatomic particle’s orientation is within the singular proton. By understanding the make-up of a proton this is taking a large step to a better understanding of subatomic interactions.
Study Analysis.
The purpose behind this project is to understand the interaction between D0 mesons and pi mesons in the collision sprays, particle explosion, following electron-proton interactions. The neutrality of the D0 meson in this experiment establishes stark evidence for the mass of a charm quark and the use of momentum in proton-electron collisions. While the charged pion is studied because of its maximum qualifications because of the quantity of pi mesons within a proton. We sought to answer the question “How does an increased Q2 impact the correlation between the transverse momentum of the D0 meson related to the charged pion’s momentum in electron-proton collisions?” We predict that there is a direct relationship between transverse momentum (pτ) of the Q2 and the overall momentum of the D0 and the charged pion in electron-proton collisions. This research will allow physicists to predict how the electron-proton collisions in future colliders might take place and the energy needed to detect them.
MATERIALS AND METHODS
Creating the correlation plot between transverse momentum of the D0 meson and the charged pion.
We conducted simulations of proton-electron collisions to predict the correlation between particles and to illustrate the data that might be collected when it is built. PYTHIA 8 is a set of C++ code used by physicists across the globe to simulate large hadron collisions. Hadron being the classification for mesons and baryons which can have strong interactions. PYTHIA 8 was used to simulate proton-electron collisions in the context of the EIC project. Initially the proton energy was set to 500 GeV and the electron energy was set to 150 GeV. The parameter Q2, the interaction strength of the collision, was set to 50 GeV and then varied to 125 GeV to see the impact that the virtual photon energy might have on the transverse momentum of the particles (Table 1). In this study, each run of the simulation involved generating 1,000,000 events to ensure the statistical reliability of the results.
Table 1. Parameters for the correlation plot between the transverse momentum and the frequency of both the D0 and the charged Pion. | |
Parameters | Rest Mass (GeV) |
Proton Energy | 500 |
Electron Energy | 150 |
Virtual Photon Energy (Q2) | 50, 125 |
PYTHIA 8 collects the transverse momentum of all the particles produced in the collision spray on the XY plane and the overall energy produced through the particle as the collision takes place. The particles identified in this project include the D0, π0, π+, and π (π representing the pion).
PYTHIA 8 collects the frequency of the particles found in the collision. The frequency is then used to calculate the correlation between the particle’s pτ and the virtual photon energy in the collision.
To create the correlation plot between the D0 meson’s pτ and the maximum, we ran a loop over all the particles in the event and identified pions and the D0 meson. When run over several events in the simulation we can analyze the identity of each particle produced. If the code was able to ‘identify the pion’ it would then record the greatest momentum of each of the pions produced in that event. That variable was then input into the plot in comparison to the D0 meson. The D0 meson did not need this same maximum code procedure because there is primarily one D0 that is observed within one proton based on the amount of charm quarks that exist within protons.
To understand the distance between the different particles being analyzed Eq.1 was used to calculate the distance between the D0 and the pion in the transverse plane (XY). R represents the distance between the two particles in ŋ, macroscopic cross section.
\[R=\sqrt{\left(\oint 2,1 – \oint 2,1\right)+\left(\eta 2,1 – \eta 2,1\right)}\tag{1}\]
RESULTS.
Particle balance within the Proton.
The first simulation was run with the electron energy at 150 GeV and the proton energy at 500 GeV. The Q2 was set at 50 GeV. As the number of events increases the number of up quarks also increases (Fig. 2). The particles necessary to track the correlation for this study are the up quark and the charm quark because they are within the desired mesons.
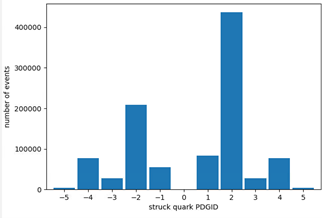
It was observed that most of the flavors, types of quarks, are contained in the 2 which is the assigned number for the up quark. Figure 2 shows less antiup quarks than up quarks. The overabundance of up quarks exists because of the existence of the positively charged proton. For the proton to exist and be positive there must be more quarks than their respective antiquarks.
Correlation between Transverse Momentum of the D0 meson and the Charged Pion Q2
There is a direct correlation between the transverse momentum of the D0 meson and the transverse momentum of the Pion. The mass of the D0 meson is higher than the pion which aligns with the mass that the D0 meson (1864.83 MeV) has compared to the pion (139.57 MeV). However, within the simulations when the Q2 (virtual photon energy) is increased the likelihood that the D0 will have a greater transverse momentum than that of the maximum pion will increase.
This spread shows where the D0’s pτ and the Pion’s pτ converge. The darker blue is going to be the depiction of where there was the lowest frequency. In the graph momentums that occur most often would be at around 4 GeV for the D0 compared to the pτ = 1.5 GeV seen by the pion. As depicted above in the first plot, the spread of the data seems to be a lot smaller compared to the spread of the second graph. While in the second graph there is a wider spread with the lightest frequency ranging between 4 and 6 GeV for the D0 and 1.5 to 3 GeV for the Pion pτ. This proves that the higher the pτ the closer into the proton can be seen. This makes sense because the more force we put behind the photon breaking up the proton the more particles we can see within the proton. As the Q2 (virtual photon energy) increases, the frequency of the pion directly increases as well.
DISCUSSION.
As mentioned before the D0 is made up of a charm quark and an antiup quark. Throughout this project the simulations looked for the momentum of the charm quarks in the system. The transverse momentum of the D0 meson exceeds that of the maximum pion pτ produced in the event. Based on this observation the momentum of the pion particle can be approximated using the momentum of the D0 meson. If it is known that the mass of the charm quark is manipulating that of smaller particles within the pion the predicted momentum of the pion can be found with the known momentum of the D0 in theory.
The spread in Fig 3A is more varied than that of Fig 3B as seen by the excess of green towards the outer edges. The Q2 is the virtual photon energy that is emitted by the electron (the overall energy in the collision). The results mentioned above convey that as the energy in the collision increases (Q2) the momentum of the D0 also increases. The charm quark takes momentum in the collision from the pion. However, the overall energy put into the collision didn’t change. This consistency explains the relationships that exist between larger hadronic particles like the charm quark and smaller particles like the pion which includes an up quark and an antidown quark.
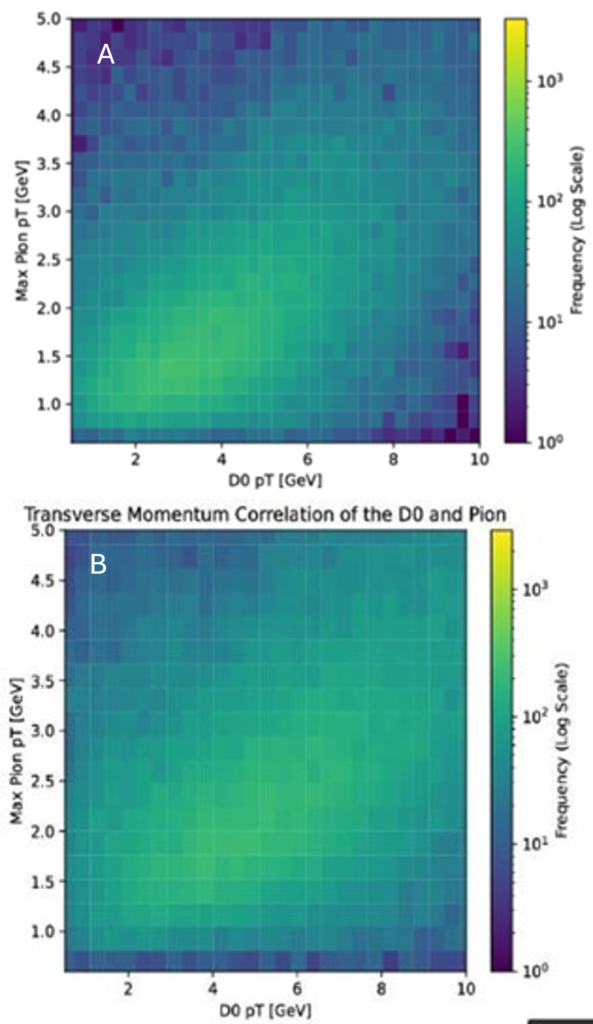
In these high energy collisions, there are many particles that exit from the proton and denature into more stable particles. This study can help understand the structure of matter inside of the proton. Because the proton is a dense particle with lots of quarks and gluons the more energy put into breaking it open (photon energy) more particles will be seen in the collision spray which helps us see further into the proton. Since the D0 meson’s momentum is always greater than the Pion’s, we can align this with what is to be expected from the internal structure of the proton. This leads to the expectation that the larger the mass of a meson, the more momentum they are going to have in relation to the amount of energy in the collision based on the energy put into the system through the virtual photon (Q2).
The dead-cone effect is a theory from the 1960s that said when a particle moving through space after a particle collision is emitting gluons there will be a space directly in front of the particle in the shape of a cone [1]. This conclusion is very similar to findings of a similar experiment based on the dead-cone effect. The experimenters found that as the mass of larger quarks increased the size of their own dead-cone diameter also increased.
The dead-cone effect further proves the results exemplified in this experiment because of the increase in mass. As the mass of the particle increases the corresponding dead-cone effect also increases [1]. It was found in this experiment that the more energy carried by the particle the more momentum will be monopolized from smaller particles.
There do exist some limitations to the experiment at hand. The simulations explained only focused on two specific particles when there are thousands of particles that can exit from a proton. Like that the study conducted was a correlational study which can’t explain a direct causational relationship between particle energy and overall momentum.
Future Directions.
As mentioned earlier, future research is needed to establish this relationship with similar and different types of particles. One example of a future particle could be the Lambda Baryon. This particle is made up of an up, down, and a strange quark. This particle would be a further step into understanding this relationship because it is made up of three positive particles while the D0 and the pion are only made up of two quarks and each includes an antiparticle as well. By understanding this relationship exists with a particle like the Lambda Baryon the relationship could expand to larger energy particles to further explain the behavior of a proton.
As these simulations are being run prior to the creation of the EIC, all these conclusions are going to be compared to the data that will be collected by the collider when collisions begin in 2033. Once these collisions are up and running, data will be collected based on the types of collisions that take place. One common question that comes along with the EIC is why does it need a lot of data to make its physics conclusion? The answer to this question lies in the fact that it takes multiple tries to get a collision head on. As mentioned before, the number of times that the electron’s photon hits the proton head on is very rare so the collider will run for a long time. All this to say that the EIC is a large step in scientific innovation and will take a big step forward for the entire scientific community.
ACKNOWLEDGMENTS.
I would like to give a big thank you to the School for Science and Math at Vanderbilt for providing this opportunity to me. I would like to give a shout out to my Instructor Dr. Rebekah Stanton and my PI Dr. Raghav for all the hours of help they provided me.
REFERENCES
- Acharya et al., “Direct observation of the dead-cone effect in quantum chromodynamics,” Nature, 605, 440-446 (2022).
- “Electron-Ion Collider (EIC).” Accessed: 05, 2023. [Online]. Available: https://www.bnl.gov/eic/
- Kumar et al., “Probing anomalous couplings using di-Higgs production in electron–proton collisions,” Physics Letters B, 764, 247–253, (2017).
- “The Electron-Ion Collider: A Precision Tool for Studying the ‘Glue’ that Binds Visible Matter,” Brookhaven National Laboratory. Accessed: 05,2023.[Online].Available: https://www.bnl.gov/news-room/news.php?a=219454
- “The Large Hadron Collider | CERN.” Accessed: Sep. 05, 2023. [Online]. Available: https://home.cern/science/accelerators/large-hadron- collider
- L. Kane and M. J. Perry, “What is a quark?,” International Journal of Modern Physics. A, Particles and Fields, Gravitation, Cosmology, 30, 1530006- (2015)
Posted by buchanle on Tuesday, April 30, 2024 in May 2024.
Tags: D0, Quark, Transverse Momentum